Introduction
Identification of antigenotoxic compounds and elucidation of the mechanisms of their action deserve special attention fortheir possible significance in the protection of human health. Extensive studies have been carried out to identify potential candidates that possess antineoplastic potential or can reduce genotoxicity of environmental agents and anticancer drugs without compromising their therapeutic effects.
Thymoquinone (TQ; Fig 1) is the main bioactive constituent of the volatile oil extracted from the black seed (Nigella sativa L.). It has been early reported to be a potent free radical scavenger (Badary et al., 2003), to reduce inflammation and mediator release in a variety of disease models (Mahgoub, 2003; Woo et al., 2012), and to be antineoplastic and pro-apoptotic in osteosarcoma cells (Shoieb, et al., 2003), neoplastic keratinocytes (Gali-Muhtasib et al., 2004), and human colon cancer cell line (Gali-Muhtasib et al., 2008). It has been further demonstrated to inhibit benzo[a]pyrene (B[a]P)-induced stomach carcinogenesis in mice (Badary et al., 1999) and attenuate diethylnitrosamine induction of hepatic carcinogenesis in rats (Sayed-Ahmed et al., 2010). In our laboratories, we had previously shown that TQ protected isolated rat hepatocytes in suspension culture against tert-butylhydroperoxide (Daba and Abdel-Rahman, 1998) and ameliorated renal oxidative damage and proliferative response caused by mercuric chloride in rats (Fouda et al., 2008).

Previous reports on the antigenotoxic properties of TQ have yielded conflicting results when investigated against B[a]P-induced genotoxicity in animal cell systems: in one study, orally administered TQ has been shown to reduce B[a]P-induced chromosomal aberrations in the mouse bone marrow cells (Badary et al., 2007), while in another study, TQ has been shown to increase chromosomal aberrations and micronucleus formation in rat hepatocyte primary culture (Khader et al., 2009).
Because black seed is regarded in the Middle East as part of an overall holistic approach to health, and is thus incorporated into diets and everyday lifestyles, it was reasonable to verify the antigenotoxic activity of TQ in a human cell system. And because TQ is linked in a substantial body of literature with lymphocyte functions (Salem, 2005), we tested the compound in cultured human peripheral blood lymphocytes as a classical system of cytogenetic mutagenicity assay (Kuroda et al., 1992).
In this report, we investigated the antigenotoxic potential of TQ based on the same research scheme conducted previously on todralazine by Gasiorowski and Brokos (2000). We used two standard mutagens: B[a]P, the major substance in cigarette smoke and a well known carcinogen and mutagen (Tarantini et al., 2009) and mitomycin C (MMC), a well known micronucleus inducing clastogen (Mark et al., 1994). The hypothesized activity of TQ was evaluated with three well established short-term tests of genotoxicity, namely: the sister chromatid exchange (SCE), a commonly used sensitive and reliable cytogenetic marker; the cytokinesis-blocked micronucleus (CBMN) assay, a biomarker of chromosomal damage; and 6-thioguanine resistance (TGr) assay, a biomarker of subtle mutation in the hypoxanthine/guanine phosphoribosyl transferase (HPRT) locus. Any reduction in the frequency of these genotoxic end points gives an indication of the antigenotoxicity of a particular compound (Albertini et al., 2000).
Finally and because of the fact that genotoxicity can result from direct damage caused by a mutagen, or by activation of promutagens to their genotoxic derivatives mediated by microsomal mixed function oxidases or other enzyme systems involving the formation of free radicals (Inami and Mochizuki, 2002), we found it reasonable to examine the impact of TQ on free radical generation by cultured human granulocytes alone and in the presence of the standard mutagen B[a]P and the standard granulocyte stimulator and tumor promoter phorbol myristate acetate (PMA).
Materials and Methods
Chemicals
Thymoquinone (2-isopropyl-5-methyl-1, 4-benzoquinone), Benzo[a]pyrene (B[a]P), cytochalasin B, 5-bromodeoxyuridine (BrdU), phorbol 12-myristate 13-acetate (PMA), Histopaque 1077, Histopaque 1119 and 6-thioguanine are from Sigma-Aldrich (St. Louis, MO, USA). Mitomycin C (MMC) is from Kyowa Kirin Ltd., (Tokyo, Japan). Phytohemagglutinin M (PHA-M) and RPMI 1640 are from Gibco (Gaithersburg, MD, USA). The rest of chemicals and stains used in this study are of the highest analytical grade from Sigma-Aldrich unless otherwise stated.
Lymphocyte Isolation and Culture
Heparinized whole blood samples were collected by venipuncture from five healthy non-smoking male volunteers (aged 20—25 y) after giving informed consent. After dilution 1:1 with RPMI 1640 without serum, lymphocytes were isolated from whole blood using a density gradient centrifugation technique with Histopaque 1119 and Histopaque-1077 layers (English and Andersen, 1974). After washing twice with phosphate buffered-saline (PBS), isolated lymphocytes and granulocytes were cultured in universal containers in complete RPMI 1640 medium containing 10% fetal bovine serum, 100 units/ml penicillin, 100 µg/ml streptomycin, and 2 mM L-glutamine. Cells were stimulated to mitogenesis with phytohemagglutinin M (PHA-M; 1% v/v) and cultured for 72 h. Cell viability was determined by Trypan blue (Serva, Germany) staining and counting in hemocytometer.
Sister Chromatid Exchange (SCE)
The tested mutagen, B[a]P dissolved in DMSO, was added into cell cultures at a final concentration of 16 µM. The cultures were then treated with different concentrations of TQ ranging from 0.625, 1.25, 2.5, 5, and 10 µM dissolved in DMSO. Higher concentrations of TQ were not studied because they were shown to induce severe cell death within minutes to hours of treatment (Khader et al., 2009). Negative controls were treated with DMSO (0.25% v/v) which represents the maximum amount of solvent used. In order to observe SCEs, within each culture, cells were allowed to proliferate for two mitotic cycles in the presence of BrdU (8 µg/ml final concentration) added 24 h after the initiation of culture and for the next 48 h. Cultures were incubated and manipulated in a dark environment in order to prevent the photolysis of BrdU. The cells were harvested by centrifugation after 2 h incubation with colchicine (0.3 µg/ml final concentration), washed with hypotonic solution (0.075 mol/L KCl) at room temperature, then fixed in methanol/acetic acid (3:1) solution. Air-dried slides were stained with a modification of the fluorescent plus Giemsa technique (Wolff and Perry, 1974). Scoring was performed in a blind fashion. The SCE frequency was scored from an analysis of metaphase during the second cycle of division. Fifty metaphases per culture with all chromosomes intact were scored for SCEs.
Cell Cycle Kinetics
Cells on the 1st, 2nd, 3rd and subsequent mitotic divisions were counted. The mitotic index (MI) was obtained by enumerating number of cells in division/1000 scored cells per each experimental point. The replication index (RI), an indirect measure of cell cycle progression was calculated according to the following formula: RI = (M1+2M2+3M3)/100, where M1, M2 and M3 represent the number of cells undergoing the first, second and third divisions respectively (Lazutka, 1991).
Cytokinesis-Blocked Micronucleus Assay (CBMN)
The CBMN test was done using the cytochalasin B technique described previously by Fenech (1993). Lymphocytes were stimulated with PHA-M and incubated at 37 â—¦C. At 24 h culture, the cells were exposed to the standard micronucleus-inducing agent MMC (1µM) with or without different concentrations of TQ dissolved in DMSO. Cytochalasin B (6 µg/ml) was added at 44 h of incubation to arrest cytokinesis. Negative controls for determination of spontaneous damage were handled in the same manner, except for treatment with MMC and TQ. After a total of 72 h, cells were harvested by centrifugation, rinsed and submitted to a mild hypotonic treatment as described for SCE. After fixation, the air-dried slides were stained with Giemsa for 20 min. For each experiment, 1000 binucleated lymphocytes (BN) with well-preserved cytoplasm were scored. Micronuclei (MN) were identified according to the criteria established by Countryman and Heddle(1976). Data are reported as MN frequency (MNF) per 1000 BN for each experimental point.
Thioguanine Resistance (TGr) Test and HPRT Mutation Assay
Assay of frequencies of point mutation of the hypoxanthine/guanine phosphoribosyl transferase locus (HPRT MF) was carried out according to anti-bromodeoxyuridine (anti-BrdU) technique described by Maffei et al. (1999). The test is based on the fact that wild-type cells containing the HPRT enzyme convert 6-thioguanine (6-TG) into toxic metabolites leading to DNA arrest, while mutant cells lacking the HPRT locus can synthesize DNA and resist 6-TG. The HPRT mutant lymphocytes (TG resistant or TGr) could be identified immunocytochemically. Briefly, lymphocytes suspended in the culture medium were stored in T75 flasks at 4°C for 20 h to prevent phenocopies. After that, cells were re-suspended in fresh medium containing PHA-M and 6-TG (20 µM final concentration), then B[a]P (16 µM) and/or TQ were added to obtain the desired final concentrations. After 24 h, 8 µg/ml BrdU were added to the cultures for the next 24 h. Hypotonic treatment and fixation were performed in the same way as for SCE. For partial denaturation of DNA, cells were suspended in 0.5 N HCl and stored for 30 min at room temperature. Cells that incorporated BrdU into their DNA wereidentified immunocytochemically using a monoclonal mouse antibody able to recognize BrdU in single-stranded DNA (clone Bu20a; DAKO, Denmark). Visualization and staining were then performed according to the alkaline phosphatase—anti-alkaline phosphatase technique in which HPRT mutants stain intensively red.
Granulocyte Generated Superoxide Anion Detection
The influence of TQ on superoxide radical generation by human granulocytes in vitro was investigated using the routine nitroblue tetrazolium (NBT) reduction test described previously (Metcalf et al., 1986), both in the presence and in the absence of the standard granulocyte stimulator phorbol myristate acetate (PMA), and separately in the presence of the standard mutagen B[a]P. Briefly, granulocytes were suspended at a density of 2×106 cells/ml in PBS, pH 7.2, containing 1 mg/ml NBT (Nacalai Tesque, Inc., Kyoto, Japan), TQ and B[a]P (16 µM) or PMA (100 ng/ml). After incubation of the samples for 45 min at 37°C, the reaction was terminated and cells were washed with PBS. The NBT deposited inside the cells was then dissolved by adding 120 μl of 2 M KOH and 140 μl of DMSO with gentle shaking for 10 min at room temperature, then absorption at 570 nm was measured with a spectrophotometer (Jenway 6400, England) against cell-free DMSO reference. The effect of TQ on the level of granulocyte generated superoxide radicals in the presence of the standard mutagen B[a]P or the standard granulocyte stimulator PMA was examined.
Statistical Analysis
Data were presented as mean ± SE. Statistical significance between means was done as appropriate by Student’s t-test or one-way ANOVA, followed by Tukey test for pair-wise comparisons. P-values of 0.05 or less were considered significant.
Results
The antigenotoxic effect of TQ was assayed in three standard short-term tests in cultured human lymphocytes: the SCE test, the CBMN assay and the HPRT MF assay. The blood donors were five healthy male volunteers. The influence of TQ on the in vitro viability of lymphocytes was assessed with the standard trypan blue exclusion test. We established that TQ in the range of concentrations tested (0.625 — 10 µM) and DMSO (0.25% v/v) were non-toxic to human lymphocytes in vitro and that they were also non-toxic to the cells when present in the culture together with the standard mutagens B[a]P (16 μM) and MMC (1 μM).
SCE and Cell Kinetic Indices
Table 1 shows the data of SCE assay and cell cycle kinetics. The base line SCE value of human lymphocytes in negative control culture was 6.43 ± 0.41. In accordance with previous studies, a statistically significant increase in SCE per cell was obtained in all donors by incubation with B[a]P 16 μM (42.83 ± 4.33, P < 0.001 vs. base line). At the dose range of 1.25 — 10 μM, TQ significantly reduced the SCE frequency in positive control cultures in a dose-dependent manner with maximal reduction (73%) obtained with 5 µM (P < 0.001). To assess the effect of TQ on the cell cycle kinetics, we scored the RI and MI of cultured lymphocytes on the same slides. The base line values of RI and MI were 2.38 ± 0.051 and 2.97 ± 0.13 respectively. In the dose range examined, we could find no significant effect of TQ on the RI and MI in the negative control cultures, while exposure of the cultures to B[a]P caused a decrease in lymphocyte RI and MI by 30.6% and 41.7% respectively. However, treatments of B[a]P in combination with varying different doses of TQ resulted in a significant dose-dependent increase in these kinetic indices as compared to the B[a]P treatment alone (Table 1).
Table 1. Effect of TQ on Sister Chromatid Exchange (SCE), Replication Index (RI), and Mitotic Index (MI) Induced by B[A]P in Cultured Human Lymphocytes
MMC-Induced MN Frequencies
As may be seen in Fig 2, in the whole group of blood donors, the unchallenged base line MNF ranged from 3 x 10—3 to 13 x 10—3 with the mean and median values of 6.8 ± 2.35 x 10—3 and 5.0 x 10—3 respectively. There was no significant change in the mean MNF reported in cultures containing all concentrations of TQ alone. After addition of the standard MN inducing agent MMC, the mean MNF was significantly enhanced over the base line value (34.4 ± 5.58 x 10—3; P < 0.01). TQ exhibited antimutagenic activity by reducing MNF in the simultaneous treatment with MMC in the dose range between 5 to 10 µM with maximal reduction (59 %) observed in cultures containing 10 µM of TQ (11.08 ± 4.1 x 10—3; P < 0.05 vs.positive control).
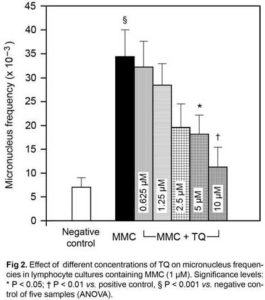
TGr Test and HPRT Mutant Frequencies
As may be shown in Fig 3, the baseline HPRT MF in negative control cultures ranged from 1.9 x 10—6 to 11.5 x 10—6 with the mean and median values of 5.3 ± 1.75 x 10—6 and 3.8 x 10—6 respectively. The mean HPRT MF did not differ significantly with all concentrations of TQ. Treatment with B[a]P increased the mean HPRT MF in positive control cultures by ~ 6 folds (31.7 ± 5.62 x 10—6; P < 0.01 vs. negative control). TQ exhibited antimutagenic activity by reducing HPRT MF in the simultaneous treatment with B[a]P in a concentration range between 2.5 to 5 µM. At the concentration of 5 µM, TQ reduced HPRT MF by 66% (10.82 ± 4.9 x 10—6; P < 0.05 vs. positive control). Although TQ at10 µM reduced HPRT MF by 48% but this reduction appeared not quite statistically significant (P = 0.0523).
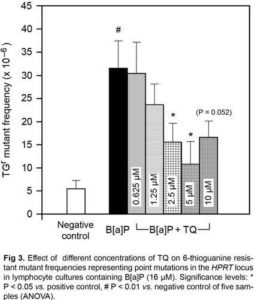
Superoxide Radicals Production by Cultured Granulocytes
As may be seen in Fig 4, treatment of granulocytes with the standard radical cascade activator PMA (100 ng/ml) and with B[a]P (16 µM) led to significant generation of superoxide radicals as monitored by NBT reduction test (P < 0.001 vs.spontaneous release control). TQ dose dependently decreased the free radical level in granulocyte samples both in the case of spontaneous release and after stimulation with PMA and with B[a]P. At the highest concentration of TQ tested (10 µM), the free radical level was lower by ~58% in the samples where TQ was only present compared to spontaneous release samples without TQ (P < 0.01). In the samples in which granulocytes were stimulated with PMA, the free radical level was lower by almost 78% in the presence of TQ (10 µM) than in the samples in which only PMA was present; whereas in the presence of B[a]P, TQ decreased the free radical level by ~ 64% in comparison with the relative control (P< 0.001 and P < 0.01 vs. relative controls respectively).
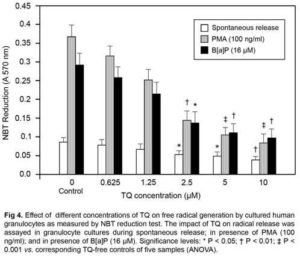
Discussion
Previous studies on genotoxic effects of TQ in animal cell systems have yielded conflicting results (Badary et al., 2007; Khader et al., 2009), and to our knowledge, there are no reports, to date, on genotoxic effects of TQ in human cell systems. In this paper we provide evidence of an antigenotoxic effect of TQ in human lymphocyte cultures subjected to two standard mutagens: an indirect acting one (B[a]P) and a direct acting one (MMC). The concentrations of the tested B[a]P (16 µM) and MMC (1 μM) were chosen based on previous studies showed that these concentrations were non-cytotoxic to lymphocytes in culture, and generated several folds increase in genotoxicity, as measured by three short-term tests (Gasiorowski and Brokos, 2000; Kocaman et al., 2013) . We used peripheral blood lymphocytes obtained from non-smoking healthy volunteers to avoid possible high levels of background mutations. Three short-term tests were used to measure different end-points of genotoxicity: the SCE test, a biomarker of chromatid rearrangements; the CBMN assay, a biomarker of clastogenic activities and chromosomal damage; and the TGr test, a biomarker of point mutations in theHPRT locus.
In the present study, TQ dose-dependently decreased the genotoxic action of the standard mutagens in the three mutagenicity tests. The antigenotoxic effect of TQ in all three tests strongly suggests that the mechanism of TQ action could be common for them. This mechanism possibly involves inhibition of mutagen processing and activation to nucleophilic derivatives; stimulation of cellular repair mechanisms; and/or activation or modulation of apoptotic pathways in genotoxically damaged cells. The detailed elucidation of the mechanisms of the antimutagenic action of TQ remains to be investigated, although we can confirm, at this moment, the first of these suggested mechanisms i.e. that involves inhibition of free radical generation and activation of promutagens into their genotoxic derivatives. This confirmation can be drawn herein both from the observed inhibitory effect of TQ alone and in combination with B[a]P or PMA upon the level of free radicals generation by human granulocytes, as assayed with the NBT reduction test, and from previous studies showing that reduction in the level of free radicals appears to be an important mechanism of the antimutagenic action of B[a]P and MMC- induced genotoxicity (Gasiorowski and Brokos, 2000; Gasiorowski et al., 2001).
It is widely known that Cytochrome P450 (CYP450) enzyme system is the major system responsible for biotransformation of xenobiotics and promutagens into their reactive nucleophilic genotoxic derivatives. This biotransformation is a complex pathway that may involve the activation of specific CYP isozymes and/or formation of free radical derivatives (Devasagayam et al., 2004; Kryston et al., 2011). The major activation of most polycyclic aromatic hydrocarbon promutagens (including B[a]P) into genotoxic products, including enhancement of oxidative stress, is mediated by CYP1A1/A2 class of CYP isozymes (Gonzalez and Gelboin, 1994). Human lymphocytes possess the same microsomal enzyme system necessary to in vitro metabolize B[a]P and other polycyclic aromatic hydrocarbons into mutagenic compounds (Goldstein and Faletto, 1993), and previous in vitro studies have reported that significant part of B[a]P wasmetabolized into a free radical form, which caused DNA adducts (Salgo et al., 1999; Gao et al., 2005). Similarly, MMC was also found to activate cellular free radical-generating systems in cultured human lymphocytes which may contribute to its main genotoxic action (Unal et al., 2012).
Taken together, the data obtained in this study on the significant inhibitory effect of TQ on B[a]P-induced free radical formation in cultured granulocytes, and the recent observation in that TQ inhibited the activity of hepatic CYP1A1/A2 isozymes involved in biotransformation of many xenobiotics into reactive genotoxic radical derivatives (Elbarbry et al., 2012) make it reasonable to conclude that one of the mechanisms by which TQ exerts its antigenotoxic activity is related to suppressing free radical derivatives responsible for DNA damage.
In this study, the maximal antigenotoxic effect of TQ in the SCE and TGr tests was obtained by the submaximal dose (5 μM), while the highest concentration (10 μM) was less effective. Significant reduction of the proliferative indices RI and MI was also noticed in lymphocytes cultures containing the highest concentration of TQ. In the CBMN test, the maximal reduction of MNF was obtained by the maximal concentration of TQ. These results appear to be attributable at least in part to the reduction of cell proliferation caused by high doses of TQ in vitro (Shoieb et al., 2003; Khader et al., 2009). In this context, it should be noted that the relations between cell proliferation and genotoxicity appear complex, although a commonly accepted opinion is that a decrease in the cell proliferation rate would facilitate detoxification and repair of damage caused by mutagenic agents (Ames et al., 1993; Tomatis, 1993). So, the low efficiency of the high dose of TQ in reduction of SCE and HPRT mutation in the TGr test, together with the maximal reduction of MNF obtained by the highest TQ concentration might be related to the reduction of RI and MI caused by the same concentration. In line with these findings, Khader et al. recently reported significant reduction of MNF and MI in rat hepatocyte primary culture with TQ concentrations higher than 10 μM while 25 μM and higher concentrations of TQ caused severe cytotoxic effects in the cultures and were lethal to hepatocytes (Khader et al., 2009).
In conclusion, the results presented in this paper confirm a dose-dependent antigenotoxic action of TQ on direct and indirect acting mutagens in vitro as proved by valid data obtained in three different end-point lymphocyte tests, and suggest that inhibition of free radical pathways could play an important role in the antigenotoxic and chemopreventive activities of TQ. This genoprotective potential remains to be evaluated at the clinical level where the combination of TQ with some clinically used anti-cancer drugs can lead to improvements in their therapeutic index and to the protection of non-tumor tissues against chemotherapy induced DNA damage.
Conflict of Interest Statement
The authors declare that there are no conflicts of interest.
References
Albertini, R. J., Anderson, D., Douglas, G. R., Hagmar, L., Hemminki, K., Merlo, F., Natarajan, A. T., Norppa, H., Shuker, D. E. G., Tice, R., Waters, M. D. & Aitio, A. (2000). “IPCS Guidelines for the Monitoring of Genotoxic Effects of Carcinogens in Humans. International Programme on Chemical Safety,” Mutation Research/Reviews in Mutation Research, 463: 111-72.
Publisher – Google Scholar
Ames, B. N., Shigenaga, M. K. & Gold, L. S. (1993). “DNA Lesions, Inducible DNA Repair, and Cell Division: Three Key Factors in Mutagenesis and Carcinogenesis,” Environmental Health Perspectives, 101: 35-44.
Publisher – Google Scholar
Badary, O. A., Abd-Ellah, M. F., El-Mahdy, M. A., Salama, S. A. & Hamada, F. M. (2007). “Anticlastogenic Activity of Thymoquinone Against Benzo(A)Pyrene in Micem,” Food and Chemical Toxicology, 45: 88-92.
Publisher – Google Scholar
Badary, O. A., Al-Shabanah, O. A., Nagi, M. N., Al-Rikabi, A. C. & Elmazar, M. M. (1999). “Inhibition of Benzo(A)Pyrene-Induced Forestomach Carcinogenesis in Mice by Thymoquinone,” European Journal of Cancer Prevention, 8:435-40.
Publisher – Google Scholar
Badary, O. A., Taha, R. A., Gamal El-Din, A. M. & Abdel-Wahab, M. H. (2003). “Thymoquinone is a Potent Superoxide Anion Scavenger,” Drug and Chemical Toxicology, 26: 87-98.
Publisher – Google Scholar
Countryman, P. I. & Heddle, J. A. (1976). “The Production of Micronuclei from Chromosome Aberration in Irradiated Cultures of Human Lymphocytes,” Mutation Research/Fundamental and Molecular Mechanisms of Mutagenesis, 41: 321—332.
Publisher – Google Scholar
Daba, M. H. & Abdel-Rahman, M. S. (1998). “Hepatoprotective Activity of Thymoquinone in Isolated Rat Hepatocytes,”Toxicology Letters, 95: 23—29.
Publisher – Google Scholar
Devasagayam, T. P. A., Tilak, J. C., Boloor, K. K., Sane, K. S., Ghaskadbi, S. S. & Lele, R. D. (2004). “Free Radicals and Antioxidants in Human Health: Current Status and Future Prospects,” Journal of Association of Physicians of India, 52: 794-804.
Publisher – Google Scholar
Elbarbry, F., Ragheb, A., Marfleet, T. & Shoker, A. (2012). “Modulation of Hepatic Drug Metabolizing Enzymes by Dietary Doses of Thymoquinone in Female New Zealand White Rabbits,” Phytotherapy Research, 26: 1726-30.
Publisher – Google Scholar
English, D. & Andersen, B. R. (1974). “Single Step Separation of Blood Cells, Granulocytes and Mononuclear Leucocytes on Discontinous Density Gradients of Ficoll-Hypaque,” Journal of Immunological Methods, 5: 246—255.
Publisher – Google Scholar
Fenech, M. (1993). “The Cytokinesis-Block Micronucleus Technique: A Detailed Description of the Method and Its Application to Genotoxicity Studies in Human Populations,” Mutation Research/Fundamental and Molecular Mechanisms of Mutagenesis, 285: 35-44.
Publisher – Google Scholar
Fouda, A.- M. M., Daba, M.- H. Y., Dahab, G. M. & Sharaf El-Din, O. A. (2008). “Thymoquinone Ameliorates Renal Oxidative Damage and Proliferative Response Induced by Mercuric Chloride in Rats,” Basic & Clinical Pharmacology & Toxicology, 103: 109 -118.
Publisher – Google Scholar
Gali-Muhtasib, H., Kuester, D., Mawrin, C., Bajbouj, K., Diestel, A., Ocker, M., Habold, C., Foltzer-Jourdainne, C., Schoenfeld, P., Peters, B., Diab-Assaf, M., Pommrich, U., Itani, W., Lippert, H., Roessner, A. & Schneider-Stock, R. (2008). “Thymoquinone Triggers Inactivation of the Stress Response Pathway Sensor CHEK1 and Contributes to Apoptosis in Colorectal Cancer Cells,” Cancer Research, 68: 5609 – 5618.
Publisher – Google Scholar
Gali-Muhtasib, H. U., Aboukheir, W., Kheir, L., Darwiche, N. & Crooks, P. (2004). “Molecular Pathway for Thymoquinone-Induced Cell Cycle Arrest and Apoptosis in Neoplastic Keratinocytes,” Anti-Cancer Drugs, 15: 389—399.
Publisher – Google Scholar
Gao, D., Luo, Y., Guevara, D., Wang, Y., Rui, M., Goldwyn, B., Lu, Y., Smith, E. C., Lebwohl, M. & Wei, H. (2005). “Benzo[A]Pyrene and Its Metabolites Combined with Ultraviolet a Synergistically Induce 8-Hydroxy-2′-Deoxyguanosine via Reactive Oxygen Species,” Free Radical Biology and Medicine, 39: 1177-83.
Publisher – Google Scholar
Gasiorowski, K. & Brokos, B. (2000). “Evaluation of Antimutagenic Effect of Todralazine in Cultured Lymphocytes,”Mutagenesis, 15: 137 – 141.
Publisher – Google Scholar
Gasiorowski, K., Brokos, B., Szyba, K. & Leszek, J. (2001). “Antimutagenic Activity of Fluphenazine in Short-Term Tests,”Mutagenesis, 16: 31 – 38.
Publisher – Google Scholar
Goldstein, J. A. & Faletto, M. B. (1993). “Advances in Mechanisms of Activation and Deactivation of Environmental Chemicals,” Environmental Health Perspectives, 100: 169-76.
Publisher – Google Scholar
Gonzalez, F. J. & Gelboin, H. V. (1994). “Role of Human Cytochromes P450 in the Metabolic Activation of Chemical Carcinogens and Toxins,” Drug Metabolism Reviews, 26: 165—183.
Publisher – Google Scholar
Inami, K. & Mochizuki, M. (2002). “Chemical Models for Cytochrome P450 as a Biomimetic Metabolic Activation System in Mutation Assays,” Mutation Research/Genetic Toxicology and Environmental Mutagenesis, 519: 133-40.
Publisher – Google Scholar
Khader, M., Bresgen, N. & Eckl, P. M. (2009). “In Vitro Toxicological Properties of Thymoquinone,” Food and Chemical Toxicology, 47: 129-33.
Publisher – Google Scholar
Kocaman, A. Y., Istifli, E. S., Büyükleyla, M., Rencüzogullari, E. & Topaktas, M. (2013). “In Vitro Evaluation of the Protective Effects of 4-Thujanol against Mitomycin-C and Cyclophosphamide-Induced Genotoxic Damage in Human Peripheral Lymphocytes,” Toxicology & Industrial Health, 29: 23 – 37.
Publisher – Google Scholar
Kryston, T. B., Georgiev, A. B., Pissis, P. & Georgakilas, A. G. (2011). “Role of Oxidative Stress and DNA Damage in Human Carcinogenesis,” Mutation Research/Fundamental and Molecular Mechanisms of Mutagenesis, 711: 193-201.
Publisher – Google Scholar
Kuroda, Y., Jain, A. K., Tezuka, H. & Kada, T. (1992). “Antimutagenicity in Cultured Mammalian Cells,” Mutation Research, 267: 201-9.
Publisher – Google Scholar
Lazutka, J. R. (1991). “Replication Index in Cultured Human Lymphocytes: Methods for Statistical Analysis and Possible Role in Genetic Toxicology,” Environmental and Molecular Mutagenesis, 17: 188-95.
Publisher – Google Scholar
Maffei, F., Vigagni, F., Norppa, H. & Hrelia, P. (1999). “Bromodeoxyuridine Labelling as an Alternative Method to Identify 6-Thioguanine-Resistant Mutant Lymphocytes in Humans,” Mutation Research/Fundamental and Molecular Mechanisms of Mutagenesis, 431: 223-31.
Publisher – Google Scholar
Mahgoub, A. A. (2003). “Thymoquinone Protects against Experimental Colitis in Rats,” Toxicology Letters, 143: 133— 43.
Publisher – Google Scholar
Mark, H. F., Naram, R., Pham, T., Shah, K., Cousens, L. P., Wiersch, C., Airall, E., Samy, M., Zolnierz, K. & Mark, R. (1994). “A Practical Cytogenetic Protocol for in Vitro Cytotoxicity and Genotoxicity Testing,” Annals of Clinical and Laboratory Science, 24: 387 – 395.
Publisher – Google Scholar
Metcalf, J., Gallin, J., Nauseef, W. & Root, R. (1986). ‘Nitroblue Tetrazolium Test,’ In Metcalf, J.A. (Ed.), Laboratory Manual of Neutrophil Function. Raven Press, New York, NY, Pp. 100—103.
Salem, M. L. (2005). “Immunomodulatory and Therapeutic Properties of the Nigella Sativa L. Seed,” International immunopharmacology, 5: 1749-70.
Publisher – Google Scholar
Salgo, M. G., Cueto, R., Winston, G. W. & Pryor, W. A. (1999). “Beta Carotene and Its Oxidation Products Have Different Effects on Microsome Mediated Binding of Benzo[A]Pyrene to DNA,” Free Radical Biology and Medicine, 26: 162-73.
Publisher – Google Scholar
Sayed-Ahmed, M. M., Aleisa, A. M., Al-Rejaie, S. S., Al-Yahya, A. A., Al-Shabanah, O. A., Hafez, M. M. & Nagi, M. N. (2010). “Thymoquinone Attenuates Diethylnitrosamine Induction of Hepatic Carcinogenesis through Antioxidant Signaling,” Oxidative Medicine and Cellular Longevity, 3: 254-261.
Publisher – Google Scholar
Shoieb, A. M., Elgayyar, M., Dudrick, P. S., Bell, J. L. & Tithof, P. K. (2003). “In Vitro Inhibition of Growth and Induction of Apoptosis in Cancer Cell Lines by Thymoquinone,” International Journal of Oncology, 22:107—113.
Publisher – Google Scholar
Tarantini, A., Maitre, A., Lefebvre, E., Marques, M., Marie, C., Ravanat, J. L. & Douki, T. (2009). “Relative Contribution of DNA Strand Breaks and DNA Adducts to the Genotoxicity of Benzo[A]Pyrene as a Pure Compound and in Complex Mixtures,” Mutation Research/Fundamental and Molecular Mechanisms of Mutagenesis, 671: 67-75.
Publisher – Google Scholar
Tomatis, L. (1993). “Cell Proliferation and Carcinogenesis: A Brief History and Current View Based on an IARC Workshop Report. International Agency for Research on Cancer,” Environmental Health Perspectives, 101: 149-51.
Publisher – Google Scholar
Unal, F., Taner, G., Yuzbasioglu, D. & Yilmaz, S. (2013). “Antigenotoxic Effect of Lipoic Acid against Mitomycin-C in Human Lymphocyte Cultures,” Cytotechnology, Nov 7. [Epub Ahead of Print]
Publisher – Google Scholar
Wolff, S. & Perrym P. (1974). “Differential Giemsa Staining of Sister Chromatids and the Study of Chromatid Exchanges without Autoradiography,” Chromosoma, 48: 341-53.
Publisher – Google Scholar
Woo, C. C., Kumar, A. P., Sethi, G. & Tan, K. H. B. (2012). “Thymoquinone: Potential Cure for Inflammatory Disorders and Cancer,” Biochemical Pharmacology, 83: 443-51.
Publisher – Google Scholar